In the last months, the scientific community welcomed the news that the first CRISPR gene therapy (Casgevy) was approved by the regulatory agencies1 around the world. This therapy is being used to treat blood disorders and sets the stage for the approval of other CRISPR-based treatments. While we wait for these, we decided to dive into the basics of this technology that have been widely used by scientists to edit DNA2 and answer several questions in biology.
The CRISPR (short name for clustered regularly interspaced short palindromic repeats) system was discovered in bacteria as a defense mechanism against viruses (yes, bacteria can be also attacked by viruses; we call them bacteriophages or simply phages). Briefly, this system works by detecting DNA from phages, cutting it into small pieces and then integrating those into the bacteria’s own DNA. If the bacteria get attacked again by the same type of the phage, they have a “memory” that allows them to find and destroy the invader (see illustration below).
To accomplish this, the CRISPR system works together with several Cas proteins that are responsible for “cutting” the phage DNA in the right way (e.g. with the specific length) but also participate in the later stages of recognizing DNA from the same type of phage during new attacks.
So, the next question was: how do the Cas proteins recognize and destroy the phage DNA? This is where another important piece of the system comes to action- the RNA (a molecule that is similar to DNA and is produced when the DNA is “read”, thus codes the same “information”). When the phage DNA is cut and pasted in the bacteria’s own DNA, it will be “read” and RNA that guides the Cas proteins will be produced. Just like a puzzle, where the different edges of one piece match another piece, the RNA will pair with the phage DNA , and will trigger the destruction of the phage DNA by the Cas proteins3.
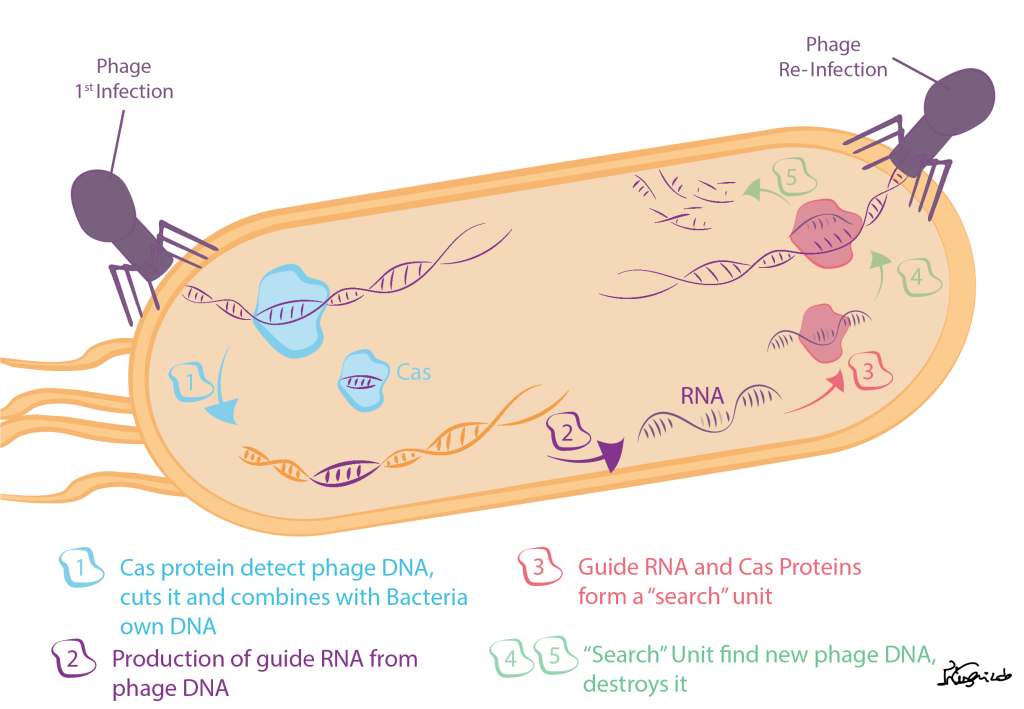
Figure 1- CRISPR molecular mechanism in bacterias.
Now, how did we learn from bacteria and start applying this mechanism to humans, allowing the creation of new therapies?
Although CRISPR can be used in different ways to treat human diseases, the current approaches use both Cas proteins (mostly a very well-studied one, Cas9) and a guide RNA. The guide RNA informs the Cas9 protein which piece of the human DNA should be cut. The way we use this system can vary from disease to disease.
In most diseases, to precisely correct DNA errors, another element is needed: the template DNA. In CRISPR therapies currently being tested, the Cas9 will first cut the part of the DNA that has errors. Then, a template DNA that does not contain those errors will be offered to the cells that will uptake this new DNA copy and repair the cut.
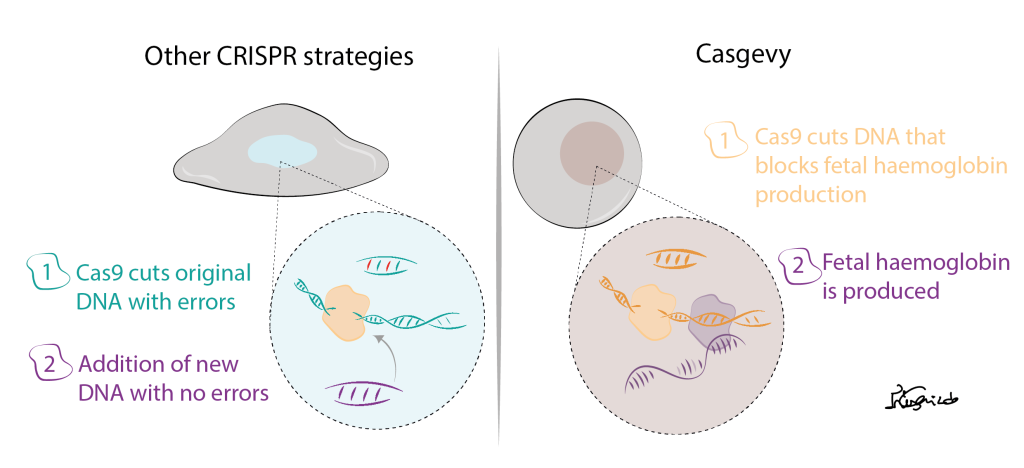
Figure 2- Strategy used for most CRISPR therapies being tested and Casgevy.
Although this is a very elegant and smart way to correct genetic diseases, scientists are still trying to show that this cut and replacement of DNA is safe for human therapies. So why is Casgevy different and why did it become the first-approved CRISPR treatment?
Well, Casgevy was created to treat patients with sickle cell disease. These patients have an error in the part of DNA that gives the cells the information how to produce haemoglobin (a protein present in our red blood cells and is needed to transport the oxygen from the lungs to the rest of our body). The trick is, in our DNA we have the information to produce 2 types of haemoglobin: the adult haemoglobin, that we have after birth and that contains errors in sickle cell disease patients and the fetal haemoglobin, the one we had when we were just a baby inside our moms’ womb. Thus, in this therapy, CRISPR is used to remove the part of the DNA that stops our cells from producing the fetal haemoglobin after birth. With this strategy, no extra DNA is added to the cells, making it a safer approach.
CRISPR is then a promising technology that has been explored to precisely correct genetic errors that give origin to diseases. While scientists have already used it broadly in the laboratory for several years, its application for human therapies is still in the beginning but holds the promise of new treatment possibilities for many people in the world.
1. Regulatory agencies- Before a new medicine enters the market it should be approved by regulatory agencies (e.g. FDA in the US, EMA in the European Union/EU) that ensure a medicine is safe to be used by people.
2. DNA- Check our previous post- “What If a Cell Was a City? – The Industry of Protein Production”
3. For more information on CRISPR biology we recommend you this page: https://innovativegenomics.org/crisprpedia/crispr-in-nature/
Comments